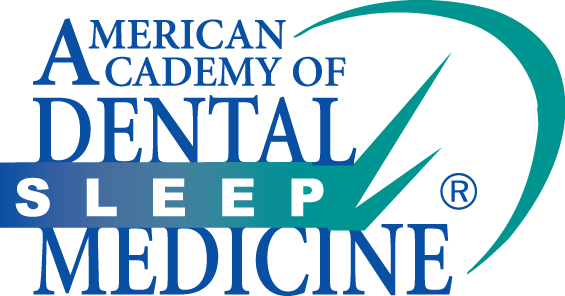
Review Article 2, Issue 3.2
Skeletal Malocclusion and Genetic Expression: An EvidenceBased Review
http://dx.doi.org/10.15331/jdsm.5720
Clarice Nishio, DDS, MSc, PhD; Nelly Huynh, PhD
Faculty of Dentistry, University of Montreal, Quebec, Canada?
ABSTRACT
Altered dentofacial morphology is an important risk factor of obstructive sleep apnea by compromising the upper airway volume. Maxillary and/or mandibular retrognathia, narrow maxilla, and long face are the most common craniofacial risk factors of sleepdisordered breathing. The etiology of dentofacial variation and malocclusion is multifactorial, which includes the influence of genetic and environmental factors acting on the units of the craniofacial complex. There is very little evidence on the reverse relationship, where changes in malocclusion could affect gene expression. The advances in human genetics and molecular biology have contributed to the identification of relevant genetic markers associated with certain skeletal malocclusions and/or dental malformations. Since some studies have observed differences between siblings, between parents/children, and between monozygotic twin pairs, this evidence suggests a significant influence of environmental factors in the development of dentofacial structures. However, the skeletal craniofacial complex has been systematically documented to be more influenced by genetic factors than the dental malocclusion. The greater the genetic component, the lower the rate of success on the outcome of orthodontic treatment. The real therapy should be an eventual modification of the gene responsible for the malocclusion; however, this is yet a theoretical proposition. The identification of major genes and determination of their biochemical action to a particular jaw discrepancy is the first approach necessary for the search of a solution. Early detection of the consequences of abnormal craniofacial development and assessment of orthodontic practices may validate the treatments used and change the natural history of pediatric obstructive sleep apnea, thereby possibly preventing or delaying the development of sleep apnea in adulthood.
Keywords:
genetics, genes, mutations, skeletal malocclusion, dental malocclusion
Citation:
Nishio C, Huynh N. Skeletal malocclusion and genetic expression: an evidence-based review. Journal of Dental Sleep Medicine 2016;3(2):57–63.
INTRODUCTION
Altered dentofacial morphology represents a major risk factor for obstructive sleep apnea by reducing the upper airway volume. Malocclusions, such as maxillary and/or mandibular retrognathia, narrow maxilla, and long face are the most common craniofacial risk factors of sleep-disordered breathing.1,2 Malocclusion is the development of a complex trait condition and relationship between both dental arches, in which occlusion has deviated from what is defined as ideal or normal occlusion. Malocclusion should not be considered as abnormal or pathological, instead as a variation of occlusion in a continuous multifactorial trait.3–7 The etiology of dentofacial variation and malocclusion is multifactorial, which includes the influence of genetic and environmental factors acting on the units of the craniofacial complex, such as bone, teeth, and muscles.5,6,8–13 However, there is very little evidence on the reverse relationship, where changes in malocclusion could affect gene expression.
DENTOFACIAL MORPHOLOGY AND GENETICS
Although new technologies have allowed the development of genetic studies, treatment objectives and therapeutic methods have not yet considered the genetic differences between individual patients.13 Among the reasons for this lack of progress are the limitations of genetic research on human populations and the different methods and concept of malocclusion used by researchers.3 Most studies on malocclusion use the Angle’s classification system, which is based simply on the dental occlusion variation of permanent first molars. While routinely used in orthodontic practice, this classification presents various deficiencies because it does not consider other vertical and transversal dental occlusion, does not evaluate the relationship of the maxilla and mandible to the cranial base, and finally, does not consider the variation among individuals.3,14 However, due to the wide consensus upon Angle’s classification among researchers, the present manuscript will use this classification system to describe similar patterns of imbalance between the jaws, but will place the term “skeletal” in front of each type of malocclusion; Class I, Class II, and Class III. Although the prevalence of these skeletal malocclusions varies according to the age, race, and population studied, it has been described to be on average 60% Class I, 35% Class II, and 5% Class III in population of western European descent.15 In the United States, Class II is present in 15% and Class III in 1% of the population.16,17 Regarding the two divisions of skeletal Class II, studies in Colombian and Iranian populations have showed that the prevalence of patients Class II, division 1 (14.9% to 24%) is higher than Class II, division 2 (3.4% to 5.9%).18,19
The recent advances in human genetics and molecular biology have contributed to the identification of relevant genetic markers associated with certain skeletal malocclusions and/or dental malformations. Among many study designs used to understand the role of genetics on malocclusion, there are studies on the skulls of ancient populations, animal models and investigation within family members and twins.4,11,12,20–25 Since some of these studies have observed notable differences between siblings, between parents and children, and even between monozygotic twin pairs, this evidence suggests a significant influence of environmental factors in the development of dentofacial structures.5,6,26,27 However, the skeletal craniofacial complex has been systematically documented to be more influenced by genetic factors than the dental malocclusion.20 Specific parts of the mandible, such as the lingual symphysis, the lateral surface of the ramus and the frontal curvature of the mandible have been described to be more susceptible to genetic control. On the other side, the antegonial notch of the mandible seems to be more influenced by environmental factors.20,22 The anterior cranial base, mandibular body length, and total and lower face heights have demonstrated highly hereditary variations.25,28 More important than to determine the degree of importance of genetics versus environmental factors in the etiology of skeletal malocclusion, is to contemplate the effect of genotype-environment interaction (epigenetic) mechanisms on the multifactorial trait in humans. Although there is not an ideal method to study the genetics of a human trait, studies using complex segregation analysis are the first step to determine if familial aggregation of a given phenotype is due to polygenes, major genes, and/or environment factors.23
The objective of this manuscript is to provide a comprehensive review of literature of the evidence for the genetic influence in the skeletal malocclusion. It is worth noting that the genetic determination on dentofacial morphology does not localize only in the bones, but it has also an influence in the neurological, muscular, and neuromuscular spheres, which have an indirect effect on the skeleton. Therefore, this review has also included studies on the genes affecting the muscular pattern of the masticatory complex. Although some dental malformations and syndromes have also been well documented to be associated with moderate to high hereditability, respectively, these two subjects will not be exploited in the present review. A systematic literature search was performed electronically in three databases (PubMed, Embase, and Medline) supplemented by a hand search and articles published from 1974 until March 2015. Search terms were combined as follows: genetics, genes, mutations, skeletal malocclusion, dental malocclusion.
Skeletal Class II, Division 1 Malocclusion
Skeletal Class II malocclusion, either division 1 or 2, is characterized by a mandibular retrusion, a maxillary protrusion, or a combination of both.20,29 Patients Class II, division 1, can also present anterior upward or downward tipping of the maxilla, steep mandibular plane angle with or without increased lower face height, and a high prevalence of transverse maxillary deficiency. The maxillary incisors have been reported to be normal or proclined, and the mandibular incisors can be in a normal, proclined, or even in a retroinclined position.15,17
Although studies have supported the concept of polygenic mode of inheritance for the skeletal Class II malocclusion, the environment has also been described to play an important role on this malocclusion.3 Adverse parafunctions, such as digital sucking, lip incompetence, protruding tongue, and nasal airway obstruction have been also associated with the induction of a clockwise rotation of the mandible and an overgrowth of the maxillary alveolar process in these patients.20,29–31
A small study of Colombian families with mandibular hypoplasia has suggested a gene candidate of this jaw size discrepancy. The human NOGGIN genes are a modulator of the bone morphogenic protein and essential for various late events in mandibular development. This study has shown that all individuals affected with mandibular hypoplasia were homozygous for the rare allele of the polymorphism rs1348322 within the NOG gene.32 Another group of genes that merits attention is the SNAIL family of zinc-finger transcription factors. These genes are important in epithelial to mesenchymal transitions and contribute to the formation of the mesoderm and the neural crest.33 The neural crest-specific deletion of Snai on a Snai2-/- background has been shown to cause craniofacial defects in mice, such as cleft palate and mandibular deficiency, indicating that these SNAIL genes may regulate the upper and lower jaw growth.34 Recently, da Fontoura et al.35 genotyped individuals with skeletal Class II for 198 single-nucleotide polymorphisms in 71 craniofacial genes and loci. They found that FGFR2 was associated with increased risk for Class II malocclusion when compared to the control group (Class I), while EDN1 was correlated with reduced risk.
Methods using the combination of principal component analysis and cluster analysis applied to data from cephalometric radiographs have provided further insight into the characterization of Class II malocclusion phenotypes. Moreno Uribe et al.17 identified seven principal components of Class II that accounted for 81% of the variation, representing a variation on mandibular rotation, maxillary incisor angulation, and mandibular length. They identified, by cluster analyses, five distinct types of Class II phenotypes.17 This study, although descriptive, gives an important evidence of the different variation of Class II traits, which indicates a significant participation of the interaction of genotype and environment on the regulation of skeletal Class II malocclusions.
Skeletal Class II, Division 2 Malocclusion
The skeletal Class II, division 2 malocclusion is characterized by a distinct and consistent clinical phenotype, which includes a combination of retroinclined incisors, deep overbite, high lip line with a lower lip trap, and high activity of the mentalis muscle. These patients often present a counter-clockwise rotation of mandibular development, prominence of the chin, and reduced lower face height.15,20 All the candidate genes for the mandibular retrognathism and deep-bite traits described in the anterior sections are associated as well with this type of division of Class II.
While some studies have described the mode of inheritance of this type of malocclusion as autosomal dominant with incomplete penetrance and variable expressivity; a polygenic model with expression of a number of genetically determined morphological traits has also been correlated to the Class II, division 2.20 This malocclusion has also been associated with higher incidence of numerous congenital tooth anomalies, such as missing teeth, peg-shaped laterals, transpositions, supernumerary teeth, and canine impactions, suggesting that genetic factors related to dental development may also play a role in the maxillomandibular size discrepancies.36
Skeletal Class III Malocclusion
Among all the types of sagittal skeletal discrepancies, the skeletal Class III is the malocclusion the most studied genetically. Class III malocclusion is caused by a deficiency of the maxilla growth, excessive mandibular growth, or a combination of both.20,23,29 It is characterized by a composite of a dentoskeletal pattern consisting of a forward positioning of the mandibular teeth in relation to the maxillary teeth and a concave profile.16 The Habsburgs, one of Europe’s royal families is an example of Mendelian inheritance of mandibular prognathism, which was observed in several generations of this family, so-called “Hapsburg jaw.” Although some authors37 consider that the X chromosome might have some role in mandibular prognathism, some other studies have verified that this trait is not X-linked since both genders are equally affected.23 It has been observed for many years that mandibular prognathism and probably maxillary deficiency contains not only a genetic component, but also an the influence of environmental factors.38 The mandibular prognathism has been reported to be a multifactorial and polygenic trait, with a threshold for expression. A study with 2,562 members from 55 families with at least one member affected with the mandibular prognathism described an autosomal dominant mode of transmission with incomplete penetrance and a heritability of 0.316.23 Taken together, these findings suggest a dominant major gene associated with the expression of mandibular prognathism and an autosomal Mendelian mode of inheritance with the influence of other genes and environmental factors.23,39 However, another study using segregation analysis of Korean families affected with the mandibular prognathism suggested that the inherited susceptibility to this malocclusion is caused by a combination of minor effects from a variety of different genes and/or environmental influence, rather than an autosomal Mendelian transmission of major genes.40Interestingly, the study of Stahl et al.41 observed a higher prevalence of genetically determined dental anomalies such as increased molar bud distance, atypical position of tooth buds, congenital hypodontia, microdontia, delayed mineralization, delayed eruption, and atypical root shape in patients affected by mandibular prognathism than in other orthodontic patients.
The Class III malocclusion associated with mandibular height and prognathism has been described with the genes ADAMTS1, ARHGAP21, GHR, Matrilin-1, EPB41, TGFB3, LTBP2, MYO1H, and KAT6B, implying that molecular pathways involved in the development of bone (TGFB3, LTBP, KAT6B) and cartilage (GHR, Matrilin-1) may be implicated in mandibular size discrepancy.16,17,40,42–47 Other candidate genes, IGF1, HOXC,COL2A1, and DUSP6 have been associated not only for with mandibular prognathism, but also with maxillary deficiency.39,48,49 Da Fontoura et al.35 described the single-nucleotide polymorphisms in FGFR2 and COL1A1 as having a higher risk for skeletal Class III, and the TBX5 gene as a reduced risk for this malocclusion.
Studies using principal component analysis and cluster analysis have been used to generate comprehensive phenotypes and to identify the most homogeneous groups of Class III subjects. Moreno Uribe et al.16 have identified 6 principal components that accounted for 81.2% of the variation, representing the variation of mandibular horizontal and vertical positions, maxillary horizontal position, and mandibular incisor angulation. In this study, the cluster model has identified 5 distinct subphenotypes of Class III malocclusion.16 Another study, using the same multivariate method, has found similar findings; 5 clusters were identified with distinct subgroups of Class III malocclusion and the 5 principal components derived from the data explained 67% of the malocclusion variation. Their results suggested that different genes might be implicated in controlling dimensions vs structures.38 These findings clearly demonstrated that Class III malocclusion exists in morphologically diverse patterns. Identifying these different phenotypes that can be related to different expressions of patient’s genotype may assist in future genetic analyses, such as genotyping and linkage studies.
Transversal Skeletal Malocclusion
The lack of transversal maxilla development and greater dental crowding have been associated with a polygenic multifactorial regulation and gene-environment interaction.20 Cutroneo et al.50 studied the integrin expression in masseter muscle specimens of severe Class III surgical patients with unilateral posterior cross bite of two or more posterior teeth. They remarked that the amount of integrins was significantly lower in muscle of the crossbite side than that observed in their counterpart. Their finding suggested that integrins may play a key role in the regulation of the masseter functional activity and may allow the optimization of contractile forces of this muscle. Whether the loss of regulatory effects on gene expression of these proteins will have an impact on the development of skeletal crossbite remains to be determined.Vertical Skeletal Malocclusion
Vertical skeletal malocclusions can be classified as skeletal open- or deep-bite, both presenting specific clinical characteristics. Skeletal open-bite is often associated with a negative overbite, hyper-divergence of the mandibular and palatal planes, increased anterior facial height, augmented clockwise facial growth, and proclined incisors.51 The inverse features, such as an increased vertical overlap between the upper and lower incisors, short anterior lower face height, excessive forward rotation of the mandible, horizontal palatal plane and a large gonial angle characterize the skeletal deep-bite individuals.15,52 The presence of open- or deep-bite in patients skeletal Class II and Class III are to some extent common.51,52Two candidates genes, PAX5 and ABCA4-ARHGAP29, have been associated with the vertical discrepancies ranging from skeletal deep to open bite.35 Remarkably, the ARHGAP29 gene has also been correlated with facial traits that are part of non-syndromic human cleft lip and/or palate.53
Genetic influences on the development of vertical malocclusions include heritable effects on both masticatory muscles and jaw morphology. Short and thin masseter muscles of low volume have been associated with dolichocephalic characteristics, such as open mandibular plane, a small posterior face height and an increased gonial angle. Conversely, long and thick muscles of high volume were related to brachycephalic features.54 The effect of the muscle will depend on the muscle thickness and the distribution of type I and II fibers.26 Another example on how the masticatory muscle activity can influence skeletal structures is the development of open-bite malocclusion in patients with muscular dystrophy. Inversely, increases in the size and proportion of fast-contracting type II fibers in masticatory muscle has been shown to play an important influence on the development of skeletal deep-bite malocclusion. In fact, Huh et al.9 have shown that genes for HDAC4 and KAT6B that regulate histone acetylation to modify chromatin accessibility and transcription were both expressed at levels several fold greater in the deep-bite muscle and Class III malocclusion than in the open-bite muscle and Class II. According to Desh et al.40 the association of KAT6B with mandibular prognathism can be correlated to its activation of the osteogenic transcription factor RUNX2, which is essential to bone growth and maintenance. Although they have also found a correlation of RUNX2 expression with masseter muscle type II fibre, the role of this protein in adult mature muscle remains to be elucidated.
Another study of Zebrick et al.8 demonstrated that the ACTN3 is a gene that influences muscle performance and fiber type proportions. A common nonsense mutation, R577X identified in the ACTN3 gene, results in a lack of alpha-actinin-3 protein expression. The loss of this protein has been shown to lead to smaller type II fiber diameters in masseter muscles and an increased expression of ENPP1, a negative regulator of mineralization. It has been demonstrated that the mutation ACTN3 R577X is overrepresented in patients with skeletal Class II malocclusion, while its underrepresentation is observed in subjects with deep bite malocclusion, suggesting a biological influence during bone development and that muscle differences contribute to the vertical facial variation.
Interestingly, the vertical skeletal malocclusions have been associated with certain genetically determined dental anomalies. A study on the prevalence of palatally displaced maxillary canines observed a significant occurrence in patients with deep-bite and the hypodivergent phenotype, three times greater than in control subjects. No association with any other type of sagittal skeletal malocclusion has been identified. These authors concluded that a genetic component is associated with the aetiology of the palatal displacement of maxillary canines.55 Another study on amelogenesis imperfecta (AI) has reported a frequent association with the skeletal open-bite malocclusion.56 These authors have shown that the homozygous carriers of enamelin (ENAM) mutation presented not only this dental anomaly, but also a Class II open-bite malocclusion. While some may believe that the coexistence of AI and open-bite malocclusion is the result of AI genes influencing the growth of the craniofacial skeleton, others may defend that dolichocephalic feature might be the result of the influence of modifying genes and/or environmental factors. Further studies are necessary to clarify whether the frequency occurrence of these dental disturbances in patients with vertical skeletal malocclusions is a mere coincidence or if there is in fact, a genetically aetiological association between these disorders.
Sagittal Skeletal Malocclusion
Certain proteins encoded by specific genes may indirectly play a role in the development of skeletal malocclusions. For example, the myosin heavy chain (MYH) is an important contractile protein that is encoded by a group of genes consisting of I (slow) IIa, IIb, IIx (fast), extraocular, embryonic, and neonatal genes. Under stress, such as when the masseter muscle is stretched or compressed following orthognathic surgery for Class II and Class III, respectively, the MYH expression in the fibre is able to change from one phenotype into another. Breuel et al.57 have showed significant difference in the levels of MYH8, MYH1, and FOXO3a between Class II patients and Class III patients, six months after orthognathic surgery. Most Class II subjects presented with continuing masseter atrophy following surgery and a delayed conversion of the type of fibre with the lengthening of the mandible. This evidence suggests that the genetic response to the neuromuscular adaptation of the masticatory muscle could explain the high relapse of the malocclusion following orthosurgery treatment.ROLE OF EPIGENETICS ON MALOCCLUSION
The epigenetic regulation has been suggested to play a fundamental role in the entire masticatory musculoskeletal complex during the development of a malocclusion.9 A better understanding of epigenetic factors and the mechanisms that determine gene expression is essential to clarify how genetic influences contribute to growth and to the diversity of facial phenotypes.
Among potential genes implicated in growth development, the homeobox genes are known to play a role in patterning embryonic development and considered to be the master genes of the head and face.5,58 Transcription factors, such as Hox group, muscle segment (Msx1 and Msx2), dustakkess (Dlx), orthodontical (Otx), goosecoid (Gsc), and sonic hedgehog (Shh) are responsible for activating or suppressing gene expression, which in conjunction with other genes, activate a cascade of events leading to the control of patterning and morphogenesis.59 Two major family groups of regulatory proteins, mesenchymal growth factors, bone morphogenetic proteins and the steroid/thyroid/retinoic acid, are vehicles through which the information of these genes is expressed. These mechanisms are of particular interest in research of craniofacial biology and development because they allow a better understanding of the process involved in jaw size discrepancies and/ or dysmorphogenesis.
While it is undeniable that some facial structures, such as the basic form of the mandibular body, the location of the nasal capsule, the size of the teeth and the arch shape are under direct genetic influence,5,24 it has been largely recognized that the growth and the final morphology of the dentofacial structures is determined by the impact of the environmental factors.5,26 In fact, the craniofacial size and shape are determined by a complex interaction of both genetic and environmental factors and the maxillary and mandibular discrepancies are a distinctive niche on this gene-environment dynamic spectrum. A typical example of this genetic-environment interaction is the soft tissue. Although its morphology has been considered to be primarily genetically determined, its behavior is influenced by both genetic and environment factors. For example, the environmental factors disrupt resting oral posture, which in turn, increases the vertical skeletal growth leading to a dental malocclusion. Occlusal characteristics are primarily defined by inherited muscle patterns, including the muscle patterns of the tongue.26 The occlusion and skeletal alterations are of multifactorial etiology,10 and the relative contributions of genetic and environmental may explain the phenotypic variation. Some believe that the phenotypic occlusal variations are mostly caused by environmental differences rather than due to the polygenic mode of inheritance, although there is no strong evidence for this.6,60
A study of Fraga et al.27 involving a large cohort of monozygotic twins examined the global and locus specific differences in their DNA methylation and histone acetylation. They observed that while young twins were epigenetically indistinguishable on the early years of life, older twins demonstrated remarkable differences in DNA methylation and histone modification, showing an important impact on their gene expression. These epigenetic markers were more evident in older monozygotic twins who had different lifestyles and have spent less of their lives together, evidencing the significant role of environmental factors in translating a common genotype into a diverse phenotype. Evident to say that studies in the epigenetic field are essential to allow us to have a better understanding of how different phenotypes of skeletal Class II and Class III, as earlier described, can originate from the same genotype.
The polygenic systems may have the capacity to protect developmental processes against any hostile environmental influence. However, when a substitution of deleterious genes decreases this protection beyond the level where environmental factors may be counterweighed, a skeletal developmental defect might result, such as cleft lip and palate and facial asymmetry.5 A developmental disarrangement between these genetic-environmental interactions may explain not only craniofacial abnormalities, but also can help us to better comprehend the regulation of maxillary, mandibular and tooth morphologies.
CLINICAL APPLICATIONS AND CONCLUSION
Preliminary studies suggest that orthodontic treatments may be effective for pediatric obstructive sleep apnea,61–63 thereby possibly reducing the incidence later in adulthood: up to 70% of adults with sleep apnea snored during childhood.64 Orthodontic therapy can successfully treat skeletal jaw discrepancies by modifying the direction of dentofacial growth25 and therefore, changing the phenotype of a specific morphogenetic pattern. However, the treatment success rate depends on several factors, including the contribution of gene-environmental interaction to the malocclusion and the capacity of the orthodontic and orthopaedic appliances to modify the skeletal pattern. Still, it is unknown whether it is possible to influence the skeletal bases beyond their genetically predetermined potential.22,65 The greater the genetic component, the lower the rate of success on the outcome of orthodontic treatment. For example, if the cause of a severe mandibular prognathism is primary genetic, the treatment is considered to be only palliative and an orthognathic surgery is required. The real therapy should be an eventual modification of the gene responsible for the mandibular prognathism, however this is yet a theoretical proposition. Nevertheless, the identification of major genes and determination of their biochemical action to a particular jaw discrepancy is the first approach necessary for the search of a solution.3
Further studies with randomized clinical trials on longitudinal cohorts of patients treated with different treatment approaches and also, genetic mapping and statistical techniques to family and twin data are the pathways to clarify the interaction of genotype and environment on the maxillomandibular discrepancies.12 If a precise skeletal malocclusion is influenced mostly by environmental factors, the objective would be then to identify the mainly cause and intercept the harmful influence on the normal development of the dentofacial structures.21,31 However, the challenge remains on how to determine the contribution of genetic and environmental factors in a specific skeletal malocclusion.
Early detection of the consequences of abnormal craniofacial development and assessment of orthodontic practices will validate the treatments used, establish practice parameters, and change the natural history of pediatric obstructive sleep apnea, thereby possibly preventing or delaying the development of sleep apnea in adulthood. With the advent of diagnostic techniques in the field of molecular genetics, the orthodontic treatment may take on a completely new direction. Such technological advances may open doors for the development of molecular approach to develop better strategies for the diagnostic, prevention and facilitate treatment modalities.65,66
REFERENCES
2. Lee RW, Chan AS, Grunstein RR, Cistulli PA. Craniofacial phenotyping in obstructive sleep apnea--a novel quantitative photographic approach. Sleep 2009;32:37–45.
3. Smith R, Bailit H. Problems and methods in research on the genetics of dental occlusion. Angle Orthod 1977;47:65–77.
4. Normando D, Faber J, Guerreiro J, Quintao C. Dental occlusion in a split Amazon indigenous population: genetics prevails over environment. PLoS One 2011;6:e28387.
5. Mossey P. The heritability of malocclusion: part 1--genetics, principles and terminology. Br J Orthod 1999;26:103–13.
6. Corruccini RS, Townsend GC, Richards LC, Brown T. Genetic and environmental determinants of dental occlusal variation in twins of different nationalities. Hum Biol 1990;62:353–67.
7. Chung CS, Niswander JD, Runck DW, Bilben SE, Kau MC. Genetic and epidemiologic studies of oral characteristics in Hawaii’s schoolchildren. II. Malocclusion. Am J Hum Genet 1971;23:471–95.
8. Zebrick B, Teeramongkolgul T, Nicot R, et al. ACTN3 R577X genotypes associate with Class II and deepbite malocclusions. Am J Orthod Dentofacial Orthop 2014;146:603–11.
9. Huh A, Horton M, Cuenco K, et al. Epigenetic influence of KAT6B and HDAC4 in the development of skeletal malocclusion. Am J Orthod Dentofacial Orthop 2013;144:568–76.
10. Hughes T, Thomas C, Richards L, Townsend G. A study of occlusal variation in the primary dentition of Australian twins and singletons. Arch Oral Biol 2001;46:857–64.
11. Goodman HO. Genetic parameters of dentofacial development. J Dent Res 1965;44:174–84.
12. Lovelina FD, Shastri SM, Kumar PD. Assessment of the oral health status of monozygotic and dizygotic twins - a comparative study. Oral Health Prev Dent 2012;10:135–9.
13. Meister M, Masella RS. Field of dreams. Am J Orthod Dentofacial Orthop 2003;123:352–3.
14. Roedig J, Phillips B, Morford L, et al. Comparison of BMI, AHI, and apolipoprotein E ε4 (APOE-ε4) alleles among sleep apnea patients with different skeletal classifications. J Clin Sleep Med 2014;10:397–402.
15. Proffit W, Fields H, Sarver D. Contemporary orthodontics. St. Louis, MO: Mosby Elsevier, 2013.
16. Moreno Uribe LM, Vela KC, Kummet C, Dawson DV, Southard TE. Phenotypic diversity in white adults with moderate to severe Class III malocclusion. Am J Orthod Dentofacial Orthop 2013;144:32–42.
17. Moreno Uribe LM, Howe SC, Kummet C, Vela KC, Dawson DV, Southard TE. Phenotypic diversity in white adults with moderate to severe Class II malocclusion. Am J Orthod Dentofacial Orthop 2014;145:305–16.
18. Thilander B, Pena L, Infante C, Parada SS, de Mayorga C. Prevalence of malocclusion and orthodontic treatment need in children and adolescents in Bogota, Colombia. An epidemiological study related to different stages of dental development. Eur J Orthod 2001;23:153–67.
19. Borzabadi-Farahani A, Borzabadi-Farahani A, Eslamipour F. Malocclusion and occlusal traits in an urban Iranian population. An epidemiological study of 11- to 14-year-old children. Eur J Orthod 2009;31:477–84.
20. Mossey P. The heritability of malocclusion: part 2. The influence of genetics in malocclusion. Br J Orthod 1999;26:195–203.
21. Kawala B, Antoszewska J, Necka A. Genetics or environment? A twinmethod study of malocclusions. World J Orthod 2007;8:405–10.
22. Lobb W. Craniofacial morphology and occlusal variation in monozygous and dizygous twins. Angle Orthod 1987;57:219–33.
23. Cruz RM, Krieger H, Ferreira R, Mah J, Hartsfield J Jr., Oliveira S. Major gene and multifactorial inheritance of mandibular prognathism. Am J Med Genet A 2008;146A:71–7.
24. Martha K, Zetu I, Ogodescu A, Gyergay R, Kovacs S. Study of dental and skeletal disorders in mono- and dizygotic twins. Rev Med Chir Soc Med Nat Iasi 2014;118:199–204.
25. Dudas M, Sassouni V. The hereditary components of mandibular growth, a longitudinal twin study. Angle Orthod 1973;43:314–22.
26. Mew JR. The postural basis of malocclusion: a philosophical overview. Am J Orthod Dentofacial Orthop 2004;126:729–38.
27. Fraga MF, Ballestar E, Paz MF, et al. Epigenetic differences arise during the lifetime of monozygotic twins. Proc Natl Acad Sci U S A 2005;102:10604–9.
28. Horowitz S, Osborne R, DeGeorge F. A cephalometric study of craniofacial variation in adult twins. Angle Orthod 1960;30:1–5.
29. Chou S, Tseng Y, Pan C, Chang J, Chang H. Craniofacial skeletal dysplasia of opposite-sex dizygotic twins. J Formos Med Assoc 2011;110:342–6.
30. Padure H, Negru AR, Stanciu D. The class II/1 anomaly of hereditary etiology vs. thumb-sucking etiology. J Med Life 2012;5:239–41.
31. Varrela J, Alanen P. Prevention and early treatment in orthodontics: a perspective. J Dent Res 1995;74:1436–8.
32. Gutierrez SJ, Gomez M, Rey JA, Ochoa M, Gutierrez SM, Prieto JC. Polymorphisms of the noggin gene and mandibular micrognathia: a first approximation. Acta Odontol Latinoam 2010;23:13–9.
33. Nieto MA. The snail superfamily of zinc-finger transcription factors. Nat Rev Mol Cell Biol 2002;3:155–66.
34. Murray SA, Oram KF, Gridley T. Multiple functions of Snail family genes during palate development in mice. Development 2007;134:1789–97.
35. da Fontoura CS, Miller SF, Wehby GL, et al. Candidate gene analyses of skeletal variation in malocclusion. J Dent Res 2015;94:913–20.
36. Basdra E, Kiokpasoglou M, Stellzig A. The Class II Division 2 craniofacial type is associated with numerous congenital tooth anomalies. Eur J Orthod 2000;22:529–35.
37. Thompson E, Winter R. Another family with the ‘Habsburg jaw’. J Med Genet 1988;25:838–42.
38. Bui C, King T, Proffit W, Frazier-Bowers S. Phenotypic characterization of Class III patients. Angle Orthod 2006;76:564–9.
39. Nikopensius T, Saag M, Jagomägi T, et al. A missense mutation in DUSP6 is associated with Class III malocclusion. J Dent Res 2013;92:893–98.
40. Desh H, Gray SL, Horton MJ, et al. Molecular motor MYO1C, acetyltransferase KAT6B and osteogenetic transcription factor RUNX2 expression in human masseter muscle contributes to development of malocclusion. Arch Oral Biol 2014;59:601–7.
41. Stahl F, Kopp H, Feldmann H, Grabowski R. Epidemiology of Hoffmeister’s genetically determined predisposition to disturbed development of the dentition in patients with true skeletal class III malocclusion. J Orofac Orthop 2005;66:6–19.
42. Guan X, Song Y, Ott J, et al. The ADAMTS1 Gene Is Associated with Familial Mandibular Prognathism. J Dent Res 2015;94:1196–201.
43. Tassopoulou-Fishell M, Deeley K, Harvey EM, Sciote J, Vieira AR. Genetic variation in myosin 1H contributes to mandibular prognathism. Am J Orthod Dentofacial Orthop 2012;141:51–9.
44. Xue F, Wong R, Rabie AB. Identification of SNP markers on 1p36 and association analysis of EPB41 with mandibular prognathism in a Chinese population. Arch Oral Biol 2010;55:867–72.
45. Zhou J, Lu Y, Gao X, et al. The growth hormone receptor gene is associated with mandibular height in a Chinese population. J Dent Res 2005;84:1052–6.
46. Li Q, Li X, Zhang F, Chen F. The identification of a novel locus for mandibular prognathism in the Han Chinese population. J Dent Res 2011;90:53–7.
47. Perillo L, Monsurro A, Bonci E, Torella A, Mutarelli M, Nigro V. Genetic association of ARHGAP21 gene variant with mandibular prognathism. J Dent Res 2015;94:569–76.
48. Xue F, Rabie AB, Luo G. Analysis of the association of COL2A1 and IGF-1 with mandibular prognathism in a Chinese population. Orthod Craniofac Res 2014;17:144–9.
49. Frazier-Bowers S, Rincon-Rodriguez R, Zhou J, Alexander K, Lange E. Evidence of linkage in a Hispanic cohort with a Class III dentofacial phenotype. J Dent Res 2009;88:56–60.
50. Cutroneo G, Piancino MG, Ramieri G, et al. Expression of musclespecific integrins in masseter muscle fibers during malocclusion disease. Int J Mol Med 2012;30:235–42.
51. Arriola-Guillén L, Flores-Mir C. Molar heights and incisor inclinations in adults with Class II and Class III skeletal open-bite malocclusions. Am J Orthod Dentofacial Orthop 2014;145:325–32.
52. Huang GJ, Bates SB, Ehlert AA, Whiting DP, Chen SS, Bollen AM. Stability of deep-bite correction: a systematic review. J World Fed Orthod 2012;1:e89–e96.
53. Miller S, Weinberg S, Nidey N, et al. Exploratory genotype-phenotype correlations of facial form and asymmetry in unaffected relatives of children with non-syndromic cleft lip and/or palate. J Anat 2014;224:688–709.
54. Benington P, Gardener J, Hunt N. Masseter muscle volume measured using ultrasonography and its relationship with facial morphology. Eur J Orthod 1999;21:659–70.
55. Sacerdoti R, Baccetti T. Dentoskeletal features associated with unilateral or bilateral palatal displacement of maxillary canines. Angle Orthod 2004;74:725–32.
56. Hart TC, Hart PS, Gorry MC, et al. Novel ENAM mutation responsible for autosomal recessive amelogenesis imperfecta and localised enamel defects. J Med Genet 2003;40:900–6.
57. Breuel W, Krause M, Schneider M, Harzer W. Genetic stretching factors in masseter muscle after orthognathic surgery. Br J Oral Maxillofac Surg 2013;51:530–5.
58. Zernik J, Minken C. Genetic control of bone remodeling. J Calif Dent Assoc 1992;20:14–9.
59. Thesleff I. Homeobox genes and growth factors in regulation of craniofacial and tooth morphogenesis. Acta Odontol Scand 1995;53:129–34.
60. Cassidy KM, Harris EF, Tolley EA, Keim RG. Genetic influence on dental arch form in orthodontic patients. Angle Orthod 1998;68:445–54.
61. Villa MP, Rizzoli A, Rabasco J, et al. Rapid maxillary expansion outcomes in treatment of obstructive sleep apnea in children. Sleep Med 2015;16:709–16.
62. Pirelli P, Saponara M, Guilleminault C. Rapid maxillary expansion (RME) for pediatric obstructive sleep apnea: a 12-year follow-up. Sleep Med 2015;16:933–5.
63. Pirelli P, Saponara M, Guilleminault C. Rapid maxillary expansion in children with obstructive sleep apnea syndrome. Sleep 2004;27:761–6.
64. Guilleminault C, Dement WC. Sleep apnea syndromes and related sleep disorders. In: Williams R, Karacan I, eds. Sleep apnea syndromes and related sleep disorders. AR Liss, 1978:13–6.
65. Carlson DS. Biological rationale for early treatment of dentofacial deformities. Am J Orthod Dentofacial Orthop 2002;121:554–8.
66. D’Souza RN, Dunnwald M, Frazier-Bowers S, et al. Translational genetics: advancing fronts for craniofacial health. J Dent Res 2013;92:1058–64.
SUBMISSION & CORRESPONDENCE INFORMATION
Submitted for publication December, 2015
Submitted in final revised form December, 2015
Accepted for publication January, 2016
Address correspondence to: Clarice Nishio, DDS, MSc, PhD, Faculty of Dentistry, University of Montreal, 3525 Chemin Queen-Mary, Montreal, Quebec, Canada, H3V 1H9; Tel: (514) 343-2469; Fax: (1-514) 343-2233; Email: clarice.nishio@umontreal.ca
DISCLOSURE STATEMENT
This was not an industry supported study. The authors have indicated no financial conflicts of interest.
PDF